Ceramics: Firing Clay and Flaking Stone
Kenneth E. Sassaman
“Failure with clay was more complete and more spectacular than with other forms of art. . . Any one of the old four—earth, air, fire, water—can betray you.” —A.S. Byatt, The Children’s Book
Abstract
This chapter follows from the previous chapter on earthy materials to consider the social impacts when transforming clay and rock into glass and other ceramic substances. Introduced is the concept of operational sequence, or the process by which affordances of both materials and societies are assembled and disassembled as things are made, used, and discarded. In particular, this chapter contrasts how glass-like rock, such as obsidian and flint, can be broken with human force with the firing of clay to produce true ceramics. The operational sequence for making both an Ice-Age spear point and a ceramic pot illustrates the contingent relationships of physical and social acts in making things, while also showcasing the evolutionary conditions under which ancestral humans developed the cognitive, motor, and social skills to achieve particular outcomes from an array of possibilities. The application of thermal energy to first stone and then clay introduced additional affordances, as well as constraints, that inform our understanding of the potential for ceramic materials of the future. Examining the relationship of human energy application to thermal energy application in different ceramics also provides important lessons for how we can use future ceramics to store and generate energy at lower costs and with fewer negative impacts than conventional technologies.
Introduction
Ceramics are among the premier materials that connect the distant past of our earliest Homo sapiens ancestors to our future. From the first pottery vessels of ancient China to the bone implants and fuel cells of tomorrow, ceramics have been developed to solve human problems for over 20,000 years. With such a long history of research and development, ceramics embody many impacts of materials on society. Since their beginning, ceramic materials served social needs, such as preparing a meal to share with others on a plate or decorating a place of ritual gathering with a ceramic mask. Future applications in communications, medicine, and energy production ensure that ceramics will remain integral to human societies for generations to come. As has long been the case, innovations in ceramics arise from the novel interplay of the properties of different substances—clay, temper, water, flux—and the application of heat. But they also arise from changing relationships between producers and consumers, experts and novices, and men and women. Before we delve into these sorts of issues we might first ask, what exactly is a ceramic?
What Makes Something a Ceramic Material?
That plate in your kitchen sink and tiles on your bathroom floor are most likely ceramic. So too are the insulators of your light bulbs, components of your microelectronics, the brake linings of your car, and maybe even the crown on your tooth. The wide array of applications for ceramics in our past and those of the future make it difficult to define ceramics in simple terms. We can agree that ceramics are inorganic solids composed of a combination of metals and non-metal or metalloid atoms that result in nonmetallic materials. We might add that in many cases they are a refractory, that is, a substance resistant to heat. It takes a great deal of heat to make a true ceramic, and some ancient ceramic vessels were designed to convey heat efficiently, as with the wet cooking of foods like corn and barley that required prolonged simmering to make them edible.
To sort through the array of materials broadly classified as ceramic, it is useful to distinguish between traditional and technical (or functional) forms of ceramics. Traditional ceramics include objects made from clay. A by-product of the weathering of rock, clay is a fine-grained material consisting of often alumina silicates with traces of metal oxides and organic matter. To reiterate key properties of clay (see Gillespie, “Clay”): (1) size is critical (particles must be less than two microns in size); (2) the water content of clay makes it plastic, capable of being molded while still wet; (3) dried and fired clay becomes hard and brittle; and (4) most clays shrink when dried, causing cracks to form at surfaces and requiring temper to prevent cracking. The utility of clay for humans depends on how its properties are recognized and manipulated, including combining it with other substances (e.g., water, temper), and usually subjecting it to heat.
Traditional ceramics are typically based on the firing of clay. A clay can become a ceramic only if subjected to temperatures in excess of about 1,200 degrees Celsius. The outcome is known as vitrification, or the fusing of the layers of clay together with a glass. The first potters to achieve this goal lived in ancient China, under the Han Dynasty (ca. 200 BCE–220 CE); their early porcelain was not only glassy in composition but also translucent because they used white kaolin clay. Pottery making for millennia before and since the dawn of porcelain involved the production of terra cottas, earthenwares, and other forms of subceramics, meaning they were not vitrified in the firing process because the temperature was not hot enough to permanently bond the clay layers. For example, clay could simply be sunbaked to achieve a relatively durable form for purposes such as hot-rock cooking or house construction.
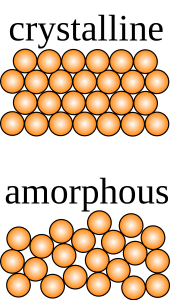
Technical forms of ceramic (functional ceramics) go well beyond the vitrification of clay to include carbides, pure oxides, and nitrides, among other materials. Ceramics can be either crystalline or amorphous, whereas glasses by definition are always amorphous (lacking long-range crystal structure). Technical ceramics can utilize their crystal structure and composition to create new, interesting properties and can also include glasses other than silicates (e.g., chalcogenides, tellurites, gallates, germanates, heavy metal oxide glass). A naturally occurring silicate glass known as obsidian that was used for millennia as raw material for stone tools is not usually considered a technical ceramic. In fact, while materials engineers consider glasses a subset of ceramics, archaeologists generally do not lump glass and ceramics together, as each has a distinct cultural history.
For the purpose of this chapter, we will focus not on the physical or chemical composition of ceramics but instead on their production and use. We are most concerned here with the transformation of matter in the manipulation of ceramics, a process that entails the harnessing of energy, which has also entailed hidden costs and unintended consequences for societies of all sorts.
Operational Sequences
A useful way to begin discussion of the impact of ceramic materials on society is to examine the production process. Here we mean production processes in general, not any particular one. All such material production processes have operational sequences, which are a series of decisions and actions that lead to intended goals. We of course follow operational sequences in many of the things we do, from cooking food, to painting a room, to repairing a bicycle tire. And we all know, from experience, what happens when a sequence is enacted out of order, especially when processes have irreversible outcomes. For example, if you add the eggs to a brownie after it’s cooked, it’s probably not palatable.
From a humanistic perspective, there is much more to operational sequences than the step-by-step procedure for getting something done. Doing work and making things involve the interplay between persons and matter, notably the bodily and social acts involved in production. Anthropologists often employ the concept of chaîne opératoire (French for operational sequence) to describe how the technical, bodily, and social acts of production are mutually dependent and collectively the basis for innovation and change. In this sense, we might say that society is made as things are made, or that production involves entanglements that reach deep into the fabric of society and its cultures (see Gillespie, “Clay”).
In their study of artifacts, archaeologists develop models of operational sequences to characterize the production of stone and bone tools, pottery, and rock art, among other things. This aids them in understanding the sociological aspects of the objects. According to archaeologist Peter Bleed, such models take two general forms.[1] Some simply describe the sequence of operations towards a predetermined goal, much like recipes in a cookbook. Others emphasize the potential for variation in sequences by looking at how technical and social issues interact dynamically. This latter approach reveals how change occurs over time and thus provides some basis for imagining how future innovations in ceramics and glass may further impact societies. Let’s take a look at the possible futures of glass by first looking back to the ancient past.
Breaking Ancestral Glass
Although our ancestors manipulated any variety of organic and inorganic substances since the beginning of human time, things made from stone comprise the oldest archaeological evidence for making things. Stones used for early tools were more durable than other substances humans modified (like wood) so by default stone tools are often the only materials we have to investigate the beginnings of human engineering. We have in the archaeological record of Africa, for instance, evidence for the making and using of flaked stone tools going back over three million years, well before the appearance of fully modern humans at about 200,000 years ago.
To call a stone tool a flaked stone tool is to indicate it was made by the removal of flakes from a parent core of rock. Flakes can be removed from cores with either percussion (knocking them off) or by applying steady pressure (pushing them off). How the stone breaks depends on a number of things, most notably what geologists call cleavage planes: the planes of relative weakness in crystalline structures. Rocks differ in their type of cleavage. Halite and galena break into cubes, calcite breaks into rhomboids, others into prisms, and so on. All of these geometric fracture tendencies tend to limit the shapes one can achieve through fracture. To achieve more complex shapes, rock that is lacking in any predetermined breakage pattern is needed.
Worldwide, rocks that lend themselves to “unnatural” breakage consist of microcrystalline quartz (silica), such as chert, flint, jasper, agate, and chalcedony, as well as a variety of lesser materials like quartzite and rhyolite.[2] In a class unto itself is obsidian, a glass-like rock that forms when felsic lava (feldspar and quartz) is cooled rapidly after being spewed from a volcano. Rapid cooling minimizes crystal growth, resulting in an amorphous glass. Obsidian like the microcrystalline materials mentioned above is very isotropic, which enables complex shape fabrication as will be discussed in the next section. With the right application of force or pressure, obsidian can be flaked to produce edges sharper than the sharpest metallic surgical scalpels.[3] In fact, obsidian surgical blades are commercially available.
Sequencing the Production of a Clovis Point
So let’s pick a traditional shape of stone tool and run through the technical operational sequence for its production. Our shape of choice is a Clovis point (Figure 3.2), a lance-shaped blade that was flaked on both sides (making it a biface) to achieve a thin, lenticular cross-section.
Next a groove or flute on the bottom of the piece needed to be fabricated in order to enable its attachment to a handle or shaft. Clovis points were made in North America from about 13,200–12,900 years ago by hunters of mammoth, mastodon, and other Ice Age creatures.[4] Modern flintknappers (persons who flake, or knap, stone) have replicated Clovis technology and attest to the high level of skill involved, particularly in removing the distinctive flutes on either face at the base, a final, risky step in the process that is successfully executed only if all other steps are followed in proper sequence.
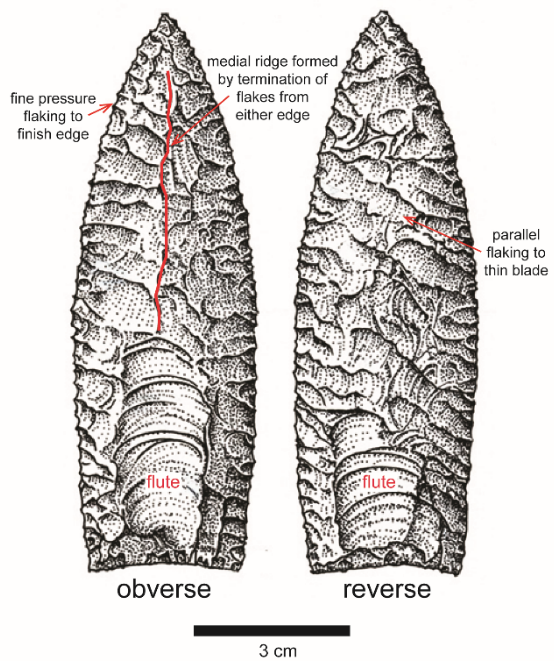
Acquire Raw Material
It goes without saying that the first step in the production of Clovis point must be the acquisition of raw material, in this case stone. But as discussed above and in the sidebar below, not any old stone will do. The technical requirements for Clovis points demand the highest-quality raw materials. North America is blessed with all sorts of quality stones for tool making (toolstones) including obsidian, but geological sources are scattered widely across the continent and some locations that were excellent for hunting and dwelling were devoid of stone. Thus, for Clovis tool makers, getting rock meant traveling to sources, either directly from places of dwelling or in the course of moving from place to place over the year. Many of the points made by Clovis hunters were displaced from the geological sources of their raw materials by hundreds of kilometers.
Activity: Watch a video
Jeff Boudreau, Massachusetts Archaeological Society, demonstrates how to make a Clovis Point in this PBS video (https://www.youtube.com/watch?v=jRax_a8t4C4&t=7s).
- Which tools were typically used to fabricate a Clovis point?
- Was it necessary to sharpen the Clovis point?
- What was the most difficult part of fabricating a Clovis point?
Prepare Raw Material for Transport
If you have to travel far from home to get rock, you probably do not want to carry back useless material. Archaeologist Charlotte Beck and colleagues researched the costs of transporting rock from quarries to places of habitation and found that ancient toolmakers minimized their transportation costs by reducing raw nodules of rock into blanks suited to further reduction.[5] The greater the distance between source and home, they found, the greater the effort to remove rocky mass of limited value. Minimally, this involved removing the cortex or exterior layer of the rock, which is not terribly conducive to flaking, as well as protuberances and other irregularities.
How Can You Tell an Actual Stone Tool from Some Old Rock?
The late-20th-century comic strip Calvin and Hobbes occasionally featured the efforts of its human protagonist to make big discoveries by digging into the earth. The few dirty rocks Calvin found in the strip above were treasure to him, but were they artifacts—that is, objects of human modification? In the case of flaked stone tools, the evidence for human agency is distinctive. Fine-grained rocks that are isotropic or amorphous are usually shaped by removing flakes from the surfaces that converge at edges. If you apply a force to an edge you drive off a flake from one of more surfaces that is elongated, and potentially very thin (instead of radial in shape if the force is applied naturally toward the core). Experts in flake-stone tool manufacture (flintknappers) can manipulate the shape of the edge and angle of applied force to essentially “sculpt” the surface of a core, one flake at a time. Archaeologists are trained to recognize the attributes of controlled reduction and can therefore distinguish manufactured tools from “a few dirty rocks,” as well as the by-products of manufacture, notably the many flakes that are removed in sequence to achieve a desired end. Sure, rocks occasionally break in ways that mimic human agency—for instance, when cobbles of chert or obsidian impact other stones during a landslide. But the more steps involved in the reduction of a core, the lower the chance a particular fracture pattern could be mimicked by natural agents. For these and many more reasons, the “treasure” archaeologists find in stone tools is the capacity they have for revealing so much about human societies of the ancient past.
Prepare Core for Reduction
Once you acquire rock of acceptable quality and transport it home, it is time to shape it into a core—a somewhat cylindrical starting piece—of appropriate form and size. At this stage of reduction, the primary objective is to preform the final product, or simply “rough it out.” It will take a hammerstone or billet (i.e., an antler or bone hammer) to achieve this objective, along with good planning and motor skills. If the nodule of rock is large enough, a large flake can be struck from its surface and used as a preform or starting point for your Clovis point. Otherwise, the preform resides inside the core itself, and can be revealed only through the careful removal of multiple flakes from across the surface. This is hardly a random or haphazard process, but instead one whose successive steps are contingent upon previous steps.
Shape and Thin Biface
The contingencies of core reduction become even more critical as you approach the final shape of the intended product. Like many flaked stone knives and projectiles, Clovis points are thin in cross-section; they have to be not only because an acute edge is needed to cut and pierce animal tissue, but also because a thin cross-section is conducive to edge maintenance, resharpening the edge to enable its ongoing use and to increase the longevity of the tool. Clovis flintknappers often used an “overshot” technique to drive flakes across the entire face of a core, a decidedly tricky move. Ultimately, however, they had to leave an elevated ridge along the midline of each face of the tool in order to accommodate the bottom flutes yet to come. To do this they had to terminate flakes at the midline, halfway across the surface, an outcome best achieved by applying pressure to the edge of the tool with an antler tip or some such implement to remove thin, narrow flakes.
Remove Flutes
Now comes the fateful moment, when the distinctive flutes of Clovis points are removed from the base of the tool on either side. Fluting will only be possible if the bifacial core has been prepared to precise tolerances. It is risky business indeed, and few modern flintknappers have mastered the technique. Experiments in percussion, pressure, and even the use of levers show that flutes can be removed in a variety of ways. Most impressive perhaps is when a flintknapper holds the biface in his or her palm and drives off a flute with the free swing of a hammer.
Finalize Edges
Now the edges have to be finalized by removing small flakes along the margins from either side. This too is done with pressure, or what is known as “pressure flaking.” Holding the tool in the palm of one’s hand, an antler tine or tip is applied to the edge to remove flakes in succession, from base to tip, or tip to base. The result is an incredibly sharp edge, serrated if the tool maker desires. This same technique will be used to resharpen the tool as its edges become dull through use.
Grind Basal Margins
Before we can attach our finished product to a handle or shaft, the basal or bottom edges of the Clovis point must be ground. This will prevent the edges from tearing into the materials used to bind the tool to its handle. An abrasive stone is good for this task, perhaps the same one used to prepare edges for flaking.
Affix to Handle or Shaft
Your finished product is not terribly useful without a handle (if it’s a knife), or a shaft (if it’s a projectile). Now that you have the basal margins or bottom ground, you can fit it to a shaft of some sort, and this step requires even more materials and know-how. A split wooden handle will accept the fluted surface of your tool nicely, but there are other options available, some involving multiple parts. No matter the option chosen, you will need some sort of binding material, such as sinew from a game animal, and perhaps some type of glue. Blood works well, as does pine resin. Keep in mind that you may want to remove your Clovis point when it breaks or is spent, because that handle was likely time-consuming and costly to make. Tool maintenance and replacement are foremost concerns.
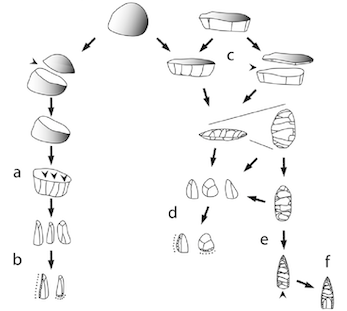
Social Implications?
We have followed an operational sequence for making a Clovis point, but so what? Could not a single individual follow this sequence alone, from beginning to end, and have a Clovis point to show for their effort? Sure, theoretically. But when we consider that Clovis points were used to dispatch mammoth and other megamammals, we understand that the application of these tools was inherently social: mammoth hunting was communal, a social affair, involving many persons, as was the processing and sharing of hundreds of pounds of meat, fat, bone marrow, hide, and other useful products from the animal.
Arguably, each step of the operational sequence for making Clovis points involved social acts. Consider that in acquiring raw material from locations hundreds of kilometers away from the manufacturing, toolmakers crossed over land occupied by others. Alternatively, rock could have been acquired in the course of regional settlement moves, what archaeologist Lewis Binford called embedded procurement.[6] In that case, acquisition was embedded in the movements of entire groups, not just individuals. Some archaeologists think that people moved entire settlements or developed territories just to be close to high quality rock.
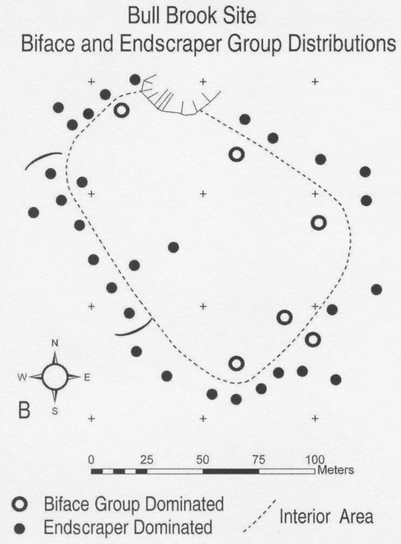
As we move on to the planned reduction of raw material into cores, the social acts involved cross-generations of toolmakers in networks of learning. Flintknapping is a nuanced skill, one that is not readily assimilated without apprenticeship and mentoring. So too is knowledge of the locations of raw materials, what anthropologists call landscape learning. The operational sequence of flaking and the final form of the Clovis point were matters of longstanding tradition, taught and maintained from generation to generation. Innovations arose that led to regional variations in how fluted points were made and used, and over time, as Clovis disappeared as a tradition and was replaced by descendent traditions, ancient knowledge was lost to change. The upshot is that technical know-how in cultures without Google and other literary forms of information sharing was transmitted socially, from expert to novice. The process of learning was situated in the relationships people had to one another; change those relationships and you impact the content and process of learning and vice versa.
And finally, in the application of fluted points for communal hunting and food sharing, core principles of society are revealed. This is exemplified best at an archaeological site in northeastern Massachusetts, at a place called Bull Brook.
From the distribution of lithic (stone) artifacts from this site, archaeologist Brian Robinson has reconstructed in detail a gathering of several dozen Paleoindian hunters and their families occupying this area 10,000–11,000 years ago.[7] Family campsites were arrayed in the large circle. The raw materials for making fluted points and a variety of other stone tools came from various places across the region, signaling a gathering of communities that otherwise spent time apart from one another. They came together to hunt caribou, a migratory herd species that was targeted for communal hunts well after mammoth and mastodons went extinct. To prepare for the hunt, toolmakers crafted many fluted points. Evidently, the risky step of fluting was shared among toolmakers. They gathered in the center of the camp circle to remove flutes, presumably under the guidance of a ritual specialist, someone who knew how to minimize risk and enhance success through many years of experience. Around the perimeter of the camp circle were clusters of flaked-stone scrapers, tools used to process hides for clothing, bedding, and perhaps tent covers. Is the spatial distinction between locations of fluting and hide working indicative of a division of labor? Likely so, and most likely a division along lines of gender, given analogs with historic-era bison hunters of the Plains (i.e., men hunted bison; women processed hides). Attributing particular tasks to particular genders has its pitfalls in archaeology, but such distinctions are nonetheless relevant to our understanding of the implications of materials on society (see Bryant, “Plastics”).
Mind, Body, and Society in Evolutionary Terms
Our rather lengthy excursion into the operational sequence of a Clovis point can be put into long-term evolutionary perspective to understand how modern humans came to be different from other species, and how human societies were impacted by transforming matter into useful products. On the first count, the mental and physical ability to make a Clovis point was underpinned by a three-million-year evolutionary history of human ancestry. If you know the classic film 2001: A Space Odyssey, you will recall the initial attempts of protohumans or advanced primates to smash bones with hammers (Figure 3.5). Lacking a tradition for tool making and thus without knowledge of an operational sequence for achieving a particular form, their results were haphazard. Still, the connection between cause (application of force) and effect (breaking bone) was apparent to these early tool makers, and, with time—and lots of trial and error—our ancestors came to understand the process of tool and weapons manufacture.
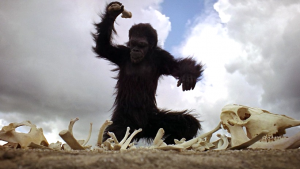
Captured in this gem of cinematic art is the process by which mind and body evolved in sync to produce a creature capable of not only applying energy (controlled force) to modify matter, but to also anticipate each step of an operational sequence to achieve desired outcomes. The cognitive and somatic developments behind this evolutionary history are beyond the scope of this chapter, but suffice to say that they arrived out of the interplay among planning, motor control, problem solving, knowledge transfer, and memory. By the time we get to the Clovis era millions of year later (only 10,000–13,000 years ago)—well beyond the evolution of fully modern humans—the level of strategic planning is impressive, albeit hidden away in the design and use of fluted points, among other tools. In this regard two aspects bear mentioning: (1) each step of the operational sequence was contingent on the prior step, and the sequence was irreversible, so the pressure was on to get it right; and (2) the costs of making a fluted point were so high that an adequate return on its investment entailed long-term maintenance and even incentives to recycle broken and worn tools into other products, a common practice at locations far from quarries.
Tools were made by transforming matter into usable material forms. But societies were also made in this process of transforming matter. Learning, sharing, cooperating and competing, territorialism, divisions of labor, gender roles, and the flows of goods, services, and personnel are all entangled in the operational sequence of making and using Clovis points. If a process can be this entangled 13,000 years ago among relatively simple, small-scale societies, imagine how much more entangled they are in complex, global-scale societies of the modern era. Conversely, perhaps we are not all that much different than these ancient toolmakers, as the history of making and using pottery suggests.
Harnessing Energy through Ceramics
As discussed earlier, a traditional ceramic is vitrified clay, essentially clay that has been transformed into glass by heat of at least 1,200 degrees Celsius. It takes a kiln or furnace to maintain temperatures this high; temperatures in an open-pit fire can exceed this threshold, but air circulation is such that average sustained temperatures rarely exceed 1,000 degrees Celsius (Figure 3.6).
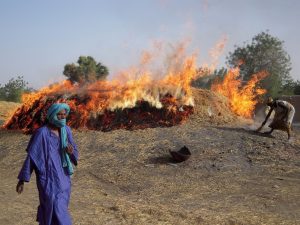
Potters using open-pit firing could sinter clay into a hard, relatively durable substance without vitrifying it, with outcomes that we classify today as subceramics. Kilns of various designs show up in several places across the globe as early as 10,000 years ago, but designs capable of vitrifying clay date to only the last 2,000 years, the earliest in China, Japan, and the Roman world.
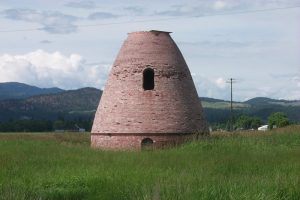
The long history of research and development from subceramics to ceramics is filled with twists and turns as potters “discovered” the latent affordances of clays, tempers, and other substances, playing off and instigating, in many cases, changes in society that inflected the demand for innovations.
Pottery and Society at the Dawn of Agriculture
The entanglements of clay and society at Çatahöyük (see Gillespie, Clay) were experienced under various circumstances by societies worldwide. Clay was particularly impactful on society when its processing accompanied the advent of agriculture. In places where domesticated grain became the staple of agricultural economies, pottery was needed to realize its nutritional value, to render wild forms of wheat, barley, and rice palatable. Most often this entailed the process of prolonged boiling or simmering. Many such grains require forty minutes or more of sustained boiling to absorb moisture and make them digestible. Pottery conducive to sustained boiling is tricky to make because one has to balance the need for thermal conductivity against the risk of thermal shock, plus pottery is generally a refractory, an insulator, not a great conductor of heat.
As we learned in Gillespie’s chapter on clay, before there was pottery at Çatahöyük there were clay balls. Similar technology was used in the American Southeast about 5,000 years ago, before clay pots were invented, and even afterwards for several centuries. The first pottery in the Southeast was designed for hot rock (and clay ball) cooking, and was thus intentionally thick-walled, to insulate internal heat. Similar technology appeared in the American Midwest a millennium later, where local communities began to consume in earnest the wild versions of weedy plants with starchy seeds, one of which (Chenopodium) is related to the quinoa that is gaining popularity today as a gluten-free grain. Thick-walled subceramics were just fine for traditional, hot-rock cooking, but if wild grains were to find a foothold in the economy, and be set on the pathway to domestication, pots that could be set directly over a fire were needed. That meant overcoming the poor thermal conductivity of clay pots.
Archaeologist David Braun documented the steps Midwestern potters took to unleash the potential of early pottery for prolonged boiling.[8] New operational sequences arose, all bent towards improving the thermal conductivity of pottery while reducing the risk of thermal shock cracking from large changes in temperature. Local clays were sufficient, but increasingly added to clay was fine quartz sand, a substance not only with decent thermal conductivity, but also with a coefficient of thermal expansion slightly greater than most clays, which left, after firing, microscopic voids in the fabric of the pottery walls that arrested cracks before they propagated. Thinner walls also challenged traditional forming techniques, which included simply molding clay into a vessel by hand, or assembling slabs into a vessel shape. A coiling technique proved effective, where walls were assembled gradually from bottom to top, like the courses of brick in a building. The walls were then compressed by paddling. The paste had to be neither too wet nor too dry to make this work. Likewise, traditional forms with angular bases and corners would no longer cut it. Jars and pots with angular bases or shoulders gave way to globular vessels; their lack of angles reduced thermal shock, and their tapered openings minimized evaporative heat loss (Figure 3.8).
Social changes attending the rise of agriculture are legion. Communities became less mobile, tethered now to patches of land they modified and to the plants they cultivated. Populations grew as both the demand for labor rose and constraints on fertility waned due to a more stationary lifestyle. Social concepts like property and inheritance emerged to foster multigenerational connections among persons, things, and land. Perceptions of time were altered to accommodate the delayed return on investments that come from farming and food storage. Greater divisions of labor appeared to meet the increasingly specialized demands of production and distribution.
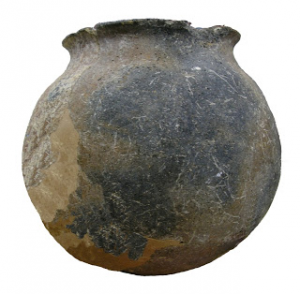
Intensify!
Above all, conditions were in place for what anthropologists call intensification, which means simply to increase production, often at increased costs per unit. Pottery was at the forefront of intensification, with numerous innovations addressing the growing demands of larger and more sedentary populations. Yes, communities had more mouths to feed. They also had other social incentives driving increased food production such as public works, military capacity, and institutions of government and religion. Eventually, with the rise of markets and commerce, the production of pottery, like so many other commodities, became specialized. Operational sequences not only became more complex—with the addition of more steps and more substances, like glazes and fluxes—but also segmented and distributed among different people, places, and schedules. Gender roles and relations were most directly affected in some cases, as in the commodification of pottery outlined below.
Key Concepts: Changing Gender Relations and the Commodification of Pottery
Visit Acoma Pueblo in New Mexico and you can buy a traditional, coil pot from one of the elder women. It will cost you, but it will be worth it. Or you could spend a lot less money on a knock-off. At Acoma, younger potters, many of whom are men, offer pots for sale that were molded, not coiled. What is remarkable from an anthropological standpoint is how the commercialization of pottery has changed gender relations. In societies worldwide, where pottery was made exclusively for domestic use, women were almost always the potters. Commercialization changed that, not only at Acoma Pueblo, but worldwide, as market economies transformed ancient ways of life. Changing gender roles in pottery making did not have to await the arrival of capitalist markets. Consider the case of Lapita pottery from Polynesia. The presumed ancestors of many Pacific cultures, people of Lapita culture began to migrate eastward across the Pacific Ocean at about 1500 BCE, reaching Tonga and Samoa by about 1000 BCE. Their pottery was a distinctive ware, decorated with repeating geometric patterns of dentate stamping. Anthropologist Yvonne Marshall believes that pots were made exclusively by women in traditional Lapita communities.[9] However, over time men got involved. Why? For Marshall, the answer traces to trade and ceremonialism. Ocean-going trade and its associated rituals were the purview of men, mostly. Increasingly, pottery production became geared towards non-domestic uses, with the more elaborate vessels funneled into exchanges controlled by men. Eventually, intensification of production broke down and ornate decoration disappeared. Throughout this period of change, plain pottery continued to be made by local communities (presumably women) for domestic uses. The Lapita case goes to show that any inducement to manufacture products beyond the level of domestic consumption introduces challenges to traditional operational sequences and their underlying social relationships. In this case, the expanding non-domestic “market” fueled demand for high-quality pots, while in the Acoma case it allowed for the development of cheap knock-offs. Despite the differences, both cases involved changes in gender roles and relations, reminding us of the impact that changing operational sequences can have on fundamental social dimensions.
Taking the long view on the history of traditional ceramics, many innovations effectively met short-term goals, and introduced unintended consequences and some new problems. The first kilns helped to concentrate heat, but they had higher construction costs and required more specialized fuels than open-pit fires. (Kilns required hardwood and other slow-burning fuels to attain and maintain temperatures in excess of 1,200 degrees Celsius). These costs were potentially offset by the longer use-lives of pots (if used in thermal applications, like cooking), but that would have dampened demand for new pots and thus thwarted the investment return of expensive infrastructure, notably the kiln itself. Over time innovations in kiln technology appeared—such as the Chinese climbing kiln (Figure 3.9), which optimized conduction—in some cases to address production demand, in others to decrease fuel costs. Clearly a major limit to production expansion for technologies involving enormous thermal energy was fuel. Until coal was introduced in the 19th-century Japanese ceramics industry, fuel consisted of wood or dung. Potting industries worldwide have contributed to local deforestation, and ultimately demand for more efficient kilns and alternative fuels, like coal and gas.
Unforeseen consequences beset the health of potters too, along with those who used their wares. Like their counterparts who manufactured gun flints centuries ago and unwittingly inhaled microscopic quartz, potters working with finely ground sand were prone to silicosis, a deadly lung disease. Likewise, British potters steeped in the tradition of lead glazing that fueled a worldwide demand for European tableware routinely suffered from lead poisoning. Add to this the collateral damage of lead exposure by consumers using pottery to process, store, and consume food. The lead threat continues today, showing how the production of something so traditional invites social interventions over labor rights, public health, and fair trade. Intensification always has its costs, direct and indirect.
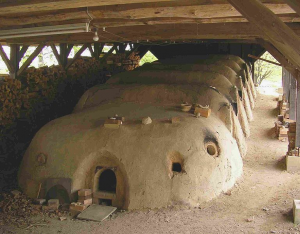
Generating and Storing Energy
In our examples of flaked stone and pottery, matter was transformed through the application of mechanical energy, mostly controlled human force. In the case of flaked stone, force and know-how was used to reduce rock from larger to smaller sizes and from amorphous to formalized shapes. In the case of pottery, force and know-how was used to assemble a variety of substances and manipulate their respective properties to form various things, finish their surfaces, and harden them with fire. This last step goes beyond human force to involve thermal energy. Applied to either rock or clay, heat altered the physical properties of substances in ways useful to people. It may seem ironic that efforts to make rock more like glass were aimed at making its breakage more predictable, while efforts to make clay more glass-like were to lessen the risk of breakage while increasing thermal conductivity.
Ceramic Fuel Cells
Is it ironic or poetic that glass-like substances offer affordances by alternately breaking and not breaking? Take this to the microscopic level of transformation and we begin to understand that putting things together and taking them apart are two sides of the same coin. That is, at the level of physiochemical change—as in the process of vitrification—energy is absorbed, stored, and released in microcosmic versions of operational sequences. Change the conditions under which these transfers of energy occur and you can produce different outcomes and create different products.
Ceramic or solid oxide fuel cells are among the more promising products that offers an alternative to more traditional electricity generation. Consider their application in domestic uses of energy. When electricity is produced at a centralized power plant and distributed to homes via a grid, the electricity has to be used immediately and the source is often the combustion of coal or natural gas. However, a fuel cell that uses hydrogen and oxygen, for instance, produces power as it is needed, locally, in the home or a refrigeration truck. It does this by using a solid oxide membrane (electrolyte) to transport oxygen ions from one side of the fuel cell to the other. When these oxygen ions react with fuel such as hydrogen or carbon monoxide, electrons are released and one produces a current.
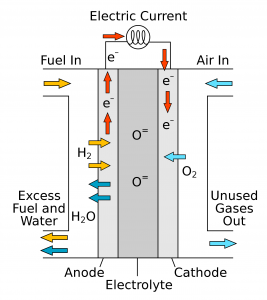
Two limitations of solid oxide fuel cells must be overcome before this technology gains a better foothold in the competition for energy production. These cells can be extremely efficient at producing electricity; however, they must operate at high temperatures in order to help with the production and transport of the oxygen ions. So the search continues for better solid oxide membrane materials to help reduce this operating temperature.
The second limitation is that fossil fuels are still involved in the process. Sure, they are not being combusted like they are in a conventional generator, but we still have the costs and impacts of creating the hydrogen of carbon monoxide fuel. What if, instead, fuel that is used to generate electricity could be generated from another source— say, the sun? Obviously, solar energy has been converted to electricity for decades now, and we all know the limitations of this technology, especially in places on the earth and at times of the year where sunlight is limited. In addition, storage of solar energy is a major limitation as it is much less efficient than the chemical storage of energy in the refined fossil fuels on which much of our energy-making infrastructure is based. So solid oxide fuel cells offer a potential way of converting solar energy to a chemical form that can be stored and used to create electricity when needed.
Getting the Most from the Sun
A promising innovation is the use of a ceramic substance, cerium dioxide, to convert solar energy into methane. The promise is nicely summarized in a short lecture by California Institute of Technology Professor Sossina Haile (https://www.youtube.com/watch?v=gSIsc7xBX3A). In this lecture, Professor Haile notes that conventional solar panels use only a portion of the spectrum of sunlight and thus fall short of their full potential for energy production. If we can capture and concentrate all the light, much like we do with a magnifying glass, we can take advantage of the full spectrum and produce lots of heat, which then can be used to drive the chemistry of fuel production. The “magic” of cerium dioxide, as Professor Haile sees it, is that it can quickly “breathe” oxygen at high temperatures. With nothing more than water, carbon dioxide, and solar heat, methane can be produced from the repetitive cycles of heating and cooling the ceramic surface. Not only is this sustainable from the standpoint of fuel production, but it helps to remove one of the greenhouse gases that is generated from combustion of fossil fuels.
Conclusion
Revealed in operational sequences are the sorts of entanglements between materials and society you read about in Gillespie’s chapter on clay. In a sense, operational sequences offer methods for analyzing entanglements by showing how bodily and social acts are entangled with materials at every step of their extraction, processing, and refinement. This is no cookbook method any more than any operational sequence is merely technical steps in a production process. The broader historical, social, and cultural contexts of any process, at any point in time, is more than a backdrop for the materials engineering going on. These social and cultural factors offer rationale, precedent, contradiction, aesthetic, opportunity, creativity, value, and more. The manipulations of glass and ceramics involved a community as a whole, and in that sense we are all materials scientists.
We saw how the making of a Clovis point was entangled with the social structure of communal hunting, and how innovations in cooking pots were bound up in the emergence and growth of farming, and how the next generation of ceramic fuel cells are constrained by an existing infrastructure and social norms for fossil fuels, but capable of decentralizing locations of production and thus making households more self-sufficient. The intrinsic value of the analysis of operational sequences is insight on change that exists in the potential relationships between materials and society. In the cases described here, heat was a common medium for changing relationships between people and things. In all cases, heat enhanced or revealed affordances that were latent to the materials being manipulated by people. But the source of heat for realizing innovation had its limits and its hidden costs, as in the higher fuel costs of kiln firing or the environmental impacts of fossil fuel combustion. With such a long history of research and development, ceramic materials of the future will continue to offer alternatives to existing technologies. And with a history of impacting societies for millennia, the production and use of ceramic materials provide ample lessons for avoiding failures and enhancing our future successes.
Discussion Questions
- Define the concept of operational sequence and explain how it encompasses social actions, as well as technical steps, in the making of things.
- How is the manufacture and use of a stone tool like a Clovis point a social act? Even though Clovis points may have been made and used by men, what impact did the technology have on women?
- What were the limitations of traditional subceramic cooking vessels in the application of sustained boiling and what did potters do to overcome those limitations?
- What is intensification and how does it impact operational sequences? What does it mean to say there are hidden costs to intensification?
- Do you see a promising future in ceramic fuel cells, and if so, what do you imagine to be the potential impact on society?
Key Terms
ceramic
operational sequence
cleavage plane
coiling
flaked stone
fluting
kiln
intensification
isotropic
subceramic
vitrification
chaîne opératoire
embedded procurement
amorphous
crystalline
Author Biography
Kenneth E. Sassaman is Hyatt and Cici Brown Professor of Florida Archaeology at the University of Florida. He earned a Ph.D. in Anthropology from the University of Massachusetts, Amherst in 1991 and has over thirty-five years of archaeological field experience in the indigenous history of the American Southeast. His most recent work centers on the challenges of climate change on the gulf coast of Florida over the past 5,000 years. He is the author of over one hundred articles and book chapters, and the author or editor of nine books, most recently The Archaeology of Ancient North America (with Tim Pauketat; Cambridge University Press, 2020).
- Peter Bleed, “Trees or Chains, Links or Branches: Conceptual Alternatives for Consideration of Stone Tool Production and Other Sequential Activities,” Journal of Archaeological Method and Theory 8 (2001): 101–27, https://doi.org/10.1023/A:1009526016167. ↵
- John C. Whittaker, Flintknapping: Making and Understanding Stone Tools (Austin, TX: Univ. of Texas Press, 1994), 65–72, http://www.worldcat.org/oclc/1041322933. ↵
- Bruce A. Buck, “Ancient Technology in Contemporary Surgery,” Western Journal of Medicine 136, no. 3 (1982): 265–69, https://www.ncbi.nlm.nih.gov/pmc/articles/PMC1273673/. ↵
- Gary Haynes, The Early Settlement of North America: The Clovis Era (Cambridge: Cambridge Univ. Press, 2002), http://www.worldcat.org/oclc/49327000. ↵
- Charlotte Beck, Amanda K. Taylor, George T. Jones, Cynthia M. Fadem, Caitlyn R. Cook, and Sara A. Millward, “Rocks are Heavy: Transport Costs and Paleolithic Quarry Behavior in the Great Basin,” Journal of Anthropological Archaeology 21, no. 4 (2002): 481–507, https://doi.org/10.1016/S0278-4165(02)00007-7. ↵
- Lewis R. Binford, “Organization and Formation Processes: Looking at Curated Technologies,” Journal of Anthropological Research 35, no. 3 (1979): 255–73, https://doi.org/10.1086/jar.35.3.3629902. ↵
- Brian S. Robinson, Jennifer C. Ort, William A. Eldridge, Adrian L. Burke, and Bertrand G. Pelletier, “Paleoindian Aggregation and Social Context at Bull Brook,” American Antiquity 74 (2009): 423–47, https://doi.org/10.1017/S0002731600048691. ↵
- David P. Braun, “Pots as Tools,” in Archaeological Hammers and Theories, eds. J. A. Moore and A. S. Keene (New York: Academic Press, 1983), 108–34, https://doi.org/10.1016/C2013-0-11196-8. ↵
- Yvonne Marshall, “Who Made the Lapita Pots?: A Case Study in Gender Archaeology," Journal of the Polynesian Society 94, no. 3 (1985): 205–33, http://www.jstor.com/stable/20705934. ↵
Feedback/Errata